Big Bang cosmic microwave background radiation: the faint afterglow of the universe’s fiery birth. It’s a whisper from the earliest moments, a snapshot of the universe when it was just 380,000 years old, a time before stars, galaxies, or even atoms as we know them existed. This ancient light, bathing the cosmos in its subtle hum, holds clues to the universe’s composition, its expansion rate, and its ultimate fate. Understanding this cosmic relic means unlocking some of the biggest mysteries surrounding our existence. Prepare to journey back to the dawn of time.
The Big Bang theory posits that the universe began from an incredibly hot, dense state and has been expanding and cooling ever since. The cosmic microwave background (CMB) is the leftover heat from this primordial fireball, a sea of photons that have been traveling through space for billions of years. Its incredibly uniform temperature, with subtle variations, provides a wealth of information about the early universe’s conditions and the processes that shaped the cosmos we observe today. Scientists meticulously analyze these minute temperature fluctuations to glean insights into the universe’s composition, its geometry, and the forces that governed its evolution.
The Big Bang Theory: Big Bang Cosmic Microwave Background
The Big Bang theory is the prevailing cosmological model for the universe. It posits that the universe originated from an extremely hot, dense state approximately 13.8 billion years ago and has been expanding and cooling ever since. While it doesn’t explain the very beginning (the singularity itself remains a mystery), it provides a remarkably successful framework for understanding the universe’s evolution from a fraction of a second after its inception to its current state.
Fundamental Principles of the Big Bang Theory
The Big Bang theory rests on several key pillars. Firstly, the universe is expanding, a fact supported by the observed redshift of distant galaxies – the farther away a galaxy, the faster it appears to be receding. Secondly, the universe is homogeneous and isotropic on large scales, meaning it looks roughly the same in all directions and from all locations. This uniformity is a crucial element in understanding the universe’s evolution. Thirdly, the cosmic microwave background radiation (CMB), a faint afterglow from the early universe, provides compelling evidence for a hot, dense early state. These observations, along with others, strongly support the Big Bang model.
Timeline of Events: From Singularity to First Atoms
The moments immediately following the initial singularity are still largely theoretical. However, within a tiny fraction of a second, the universe underwent a period of rapid expansion known as inflation. This period smoothed out initial irregularities and set the stage for the subsequent evolution. As the universe expanded and cooled, fundamental forces separated, particles formed, and eventually, the first atoms emerged. This transition from a plasma of protons, neutrons, and electrons to neutral hydrogen and helium atoms, known as recombination, occurred around 380,000 years after the Big Bang. This event is crucial because it allowed photons to travel freely, eventually forming the CMB we observe today.
Early Universe Evolution
The early universe was a vastly different place than it is now. In its initial moments, it was dominated by extremely high temperatures and energies. Gravity played a dominant role in shaping the distribution of matter. Tiny fluctuations in density, amplified by gravity, seeded the formation of the large-scale structures we see today – galaxies, galaxy clusters, and superclusters. The transition from a radiation-dominated universe to a matter-dominated universe was a significant turning point. Initially, radiation (photons) exerted a stronger influence on the universe’s expansion than matter. However, as the universe cooled, matter eventually took over, paving the way for the formation of stars and galaxies.
Epochs of the Early Universe
Epoch | Timeframe | Dominant Constituents | Key Events |
---|---|---|---|
Planck Epoch | 0 to 10-43 seconds | Unknown | Unification of fundamental forces, possibly inflation |
Grand Unification Epoch | 10-43 to 10-36 seconds | Unknown | Separation of gravity from other forces |
Electroweak Epoch | 10-36 to 10-12 seconds | Quarks, leptons, gauge bosons | Separation of electroweak force into electromagnetic and weak forces |
Quark Epoch | 10-12 to 10-6 seconds | Quarks, gluons | Quark-gluon plasma |
Hadron Epoch | 10-6 to 1 second | Protons, neutrons | Formation of protons and neutrons |
Lepton Epoch | 1 second to 10 seconds | Electrons, neutrinos | Annihilation of most positrons and anti-electrons |
Photon Epoch (Radiation-Dominated) | 10 seconds to 380,000 years | Photons, electrons, protons, nuclei | Big Bang nucleosynthesis, universe is opaque to radiation |
Recombination Epoch (Matter-Dominated) | 380,000 years | Hydrogen, helium atoms, photons | Formation of neutral atoms, universe becomes transparent to radiation (CMB forms) |
Cosmic Microwave Background Radiation (CMB)
The Cosmic Microwave Background (CMB) is arguably the most compelling piece of evidence supporting the Big Bang theory. It’s the afterglow of the universe’s fiery infancy, a faint echo whispering secrets from a time when the cosmos was a scorching, dense soup of particles. Understanding its origin and properties offers a crucial window into the universe’s earliest moments and its subsequent evolution.
CMB Origin and Connection to the Big Bang
The CMB’s origin is directly linked to the Big Bang. In the universe’s first few hundred thousand years, it was so hot and dense that photons (light particles) were constantly scattering off charged particles, preventing light from traveling freely. This era is known as the “recombination epoch,” when the universe cooled enough for protons and electrons to combine and form neutral hydrogen atoms. This event allowed photons to decouple from matter and travel unimpeded through space. These photons, redshifted significantly due to the universe’s expansion, are what we detect today as the CMB – a faint, uniform microwave radiation permeating the entire observable universe. The CMB acts as a snapshot of the universe at this crucial transition point, roughly 380,000 years after the Big Bang.
CMB Characteristics: Temperature and Spectrum
The CMB is remarkably uniform, exhibiting an almost perfect blackbody spectrum. This means its energy distribution follows a specific curve characteristic of an object in thermal equilibrium. The average temperature of the CMB is measured to be approximately 2.725 Kelvin (-270.425°C), incredibly cold, yet a testament to the universe’s cooling since the Big Bang. This uniform temperature across vast stretches of the cosmos further supports the idea of a homogeneous early universe. The near-perfect blackbody spectrum provides strong evidence for thermal equilibrium in the early universe and further validates the Big Bang model.
CMB Anisotropy and its Significance
While remarkably uniform, the CMB isn’t perfectly homogeneous. Tiny temperature fluctuations, or anisotropies, exist at the level of parts per hundred thousand. These minute variations, though seemingly insignificant, hold immense cosmological significance. They represent the seeds of large-scale structures we observe today – galaxies, galaxy clusters, and superclusters. These density fluctuations, present in the early universe, acted as gravitational wells, attracting matter and leading to the formation of the cosmic structures we see billions of years later. The study of these anisotropies, measured by satellites like COBE and WMAP and Planck, allows cosmologists to constrain cosmological parameters such as the universe’s age, geometry, and composition.
Visual Representation of CMB Temperature Fluctuations
Imagine a map of the entire sky, a celestial globe if you will. Instead of stars and constellations, this map displays subtle variations in temperature. It would appear as a seemingly uniform wash of microwave radiation, a pale, slightly mottled sky. However, upon closer inspection, one would see faint, swirling patterns – tiny hot and cold spots representing the temperature fluctuations. These fluctuations are incredibly small, only about one part in 100,000 compared to the average temperature. The hot spots are regions where the density of matter was slightly higher in the early universe, while cold spots represent regions of slightly lower density. These seemingly minuscule differences are the seeds of cosmic structure formation, the building blocks of galaxies and galaxy clusters. The overall image would be incredibly detailed, a complex tapestry of temperature variations across the entire sky, a visual testament to the universe’s tumultuous and ultimately beautiful history. The scale of these variations, from large-scale patterns to tiny details, reflects the complex interplay of gravity and the early universe’s conditions.
CMB Anisotropies and their Implications
The Cosmic Microwave Background (CMB) isn’t perfectly uniform; subtle temperature variations, known as anisotropies, are imprinted across its surface. These tiny fluctuations, only parts per million different from the average temperature of 2.725 Kelvin, hold the key to understanding the early universe’s conditions and the processes that shaped the cosmos we observe today. Analyzing these anisotropies allows cosmologists to test and refine models of the Big Bang, providing crucial insights into the universe’s composition, geometry, and evolution.
The CMB’s anisotropies aren’t random noise; they reveal a rich tapestry of information encoded in a specific pattern. This pattern, revealed through the CMB power spectrum, shows the distribution of temperature fluctuations across different angular scales. The peaks and troughs in this spectrum are not accidental; they represent the echoes of physical processes that occurred in the early universe, providing a window into its composition and dynamics.
Acoustic Peaks in the CMB Power Spectrum
The most striking feature of the CMB power spectrum is the series of acoustic peaks. These peaks arise from sound waves that propagated through the early universe’s plasma – a hot, dense soup of protons, electrons, and photons. Before recombination (when electrons combined with protons to form neutral hydrogen atoms, around 380,000 years after the Big Bang), these sound waves compressed and rarefied the plasma, leaving behind imprints on the CMB temperature distribution. The first acoustic peak corresponds to the largest sound waves that could have traversed the observable universe before recombination. Subsequent peaks represent higher-order harmonics of these waves, each revealing information about the universe’s density and geometry. The precise positions and amplitudes of these peaks provide stringent constraints on cosmological parameters like the density of baryonic matter (ordinary matter made of protons and neutrons), dark matter, and dark energy. For instance, the height of the first peak is directly related to the density of baryons. Deviations from the predicted peak heights would suggest a different cosmological model.
Types of CMB Anisotropies and their Origins
Understanding the different types of anisotropies requires considering their origins and the physical processes that shaped them. These anisotropies aren’t simply random; they are structured and informative.
- Primary Anisotropies: These anisotropies originated before recombination, imprinted on the CMB as density fluctuations in the early universe. They reflect the initial conditions of the universe, and their distribution is crucial for understanding the seeds of large-scale structure formation. The acoustic peaks are a prime example of primary anisotropies.
- Secondary Anisotropies: These anisotropies arose after recombination, due to interactions of the CMB photons with matter and gravitational fields. Examples include the Sunyaev-Zel’dovich effect (scattering of CMB photons by hot electrons in galaxy clusters, causing a temperature increase), gravitational lensing (distortion of CMB photons’ paths by intervening mass), and the Rees-Sciama effect (gravitational interaction of CMB photons with evolving large-scale structures).
The relative contributions of primary and secondary anisotropies to the observed CMB temperature fluctuations are carefully analyzed to disentangle the effects of early universe physics from later-stage processes. For example, the Sunyaev-Zel’dovich effect can be used to identify galaxy clusters, even if they are too distant to be observed directly through their light. This demonstrates the synergistic relationship between different CMB anisotropies and other cosmological observations.
CMB and Cosmological Parameters
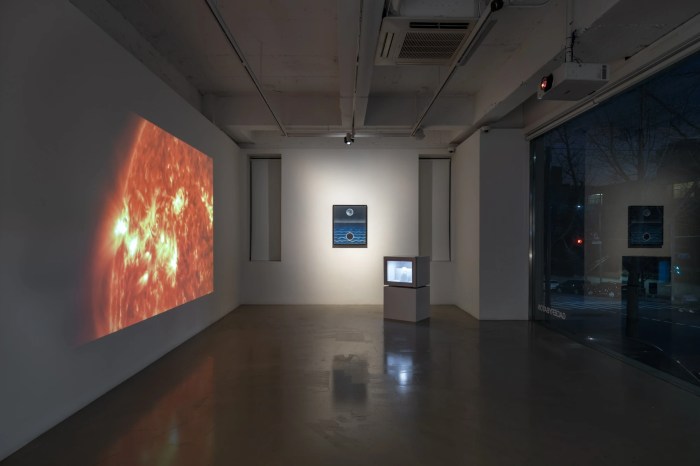
Source: cloudinary.com
The Cosmic Microwave Background (CMB) isn’t just a relic of the early universe; it’s a treasure trove of information about the cosmos’s fundamental properties. By meticulously analyzing its subtle temperature fluctuations, cosmologists can constrain – meaning to determine the range of possible values for – key cosmological parameters that govern the universe’s evolution and large-scale structure. These parameters paint a picture of the universe’s composition, geometry, and history, allowing us to test and refine our cosmological models.
The CMB’s significance lies in its ability to act as a snapshot of the universe at a very young age, approximately 380,000 years after the Big Bang. The tiny temperature variations in the CMB, known as anisotropies, reflect the density fluctuations present at that epoch. These fluctuations acted as seeds for the formation of galaxies and large-scale structures we observe today. Therefore, studying the CMB provides a direct link between the early universe and its current state.
CMB Constraints on Cosmological Parameters
Measurements of the CMB’s temperature anisotropies, particularly their power spectrum (a plot showing the amplitude of fluctuations at different angular scales), allow scientists to determine the values of several crucial cosmological parameters. These parameters, when combined with other observations, provide a comprehensive cosmological model that accurately describes the universe’s evolution. For instance, the detailed shape of the CMB power spectrum is highly sensitive to the density of dark matter and dark energy, the universe’s expansion rate (represented by the Hubble constant), and its overall geometry (flat, curved, etc.). Disagreements between different methods of measuring the Hubble constant, for example, highlight the importance of CMB data in refining our understanding. Discrepancies between CMB-derived parameters and those obtained through other means often point to areas where our cosmological models need improvement or further investigation.
CMB and the Geometry of the Universe
The geometry of the universe – whether it’s flat, positively curved (like a sphere), or negatively curved (like a saddle) – significantly influences the patterns observed in the CMB. A flat universe, predicted by the standard cosmological model and supported by CMB observations, produces a characteristic pattern in the CMB power spectrum. Deviations from this pattern would indicate a non-flat universe. The precise angular scale at which certain acoustic peaks appear in the CMB power spectrum is directly related to the universe’s curvature. This is because the curvature affects the distance light travels and, consequently, the size of the regions that appear in the CMB. Precise measurements from the Planck satellite strongly support a flat universe, consistent with the inflationary model.
CMB and the Inflationary Model
The incredibly uniform temperature of the CMB across the entire sky is a profound puzzle. Different regions of the observable universe were causally disconnected in the early universe, meaning they couldn’t have interacted to equalize their temperatures. Inflation, a period of extremely rapid expansion in the very early universe, elegantly solves this problem. During inflation, the universe expanded so rapidly that even causally disconnected regions were brought into thermal equilibrium, leading to the remarkably uniform CMB we observe. Furthermore, the tiny temperature fluctuations observed in the CMB are believed to be quantum fluctuations that were stretched and amplified during inflation. The specific pattern of these fluctuations, as revealed by the CMB power spectrum, provides strong support for the inflationary model and constrains the energy scale and duration of inflation. Predictions made by inflationary models, regarding the amplitude and distribution of these fluctuations, are remarkably consistent with the observed CMB data.
Table of Cosmological Parameters from CMB Observations
Parameter | Symbol | Measured Value (Approximate) | Significance |
---|---|---|---|
Hubble Constant | H0 | 67.4 ± 0.5 km/s/Mpc (Planck 2018) | Describes the rate of the universe’s expansion. Discrepancies with other measurements are actively being investigated. |
Baryon Density | Ωbh2 | 0.0224 ± 0.0001 (Planck 2018) | Represents the density of ordinary matter (protons and neutrons). Crucial for understanding star and galaxy formation. |
Dark Matter Density | Ωch2 | 0.120 ± 0.001 (Planck 2018) | Represents the density of dark matter, a mysterious substance that makes up a significant portion of the universe’s mass. |
Dark Energy Density | ΩΛ | 0.685 ± 0.007 (Planck 2018) | Represents the density of dark energy, a mysterious force driving the accelerated expansion of the universe. |
Challenges and Future Research
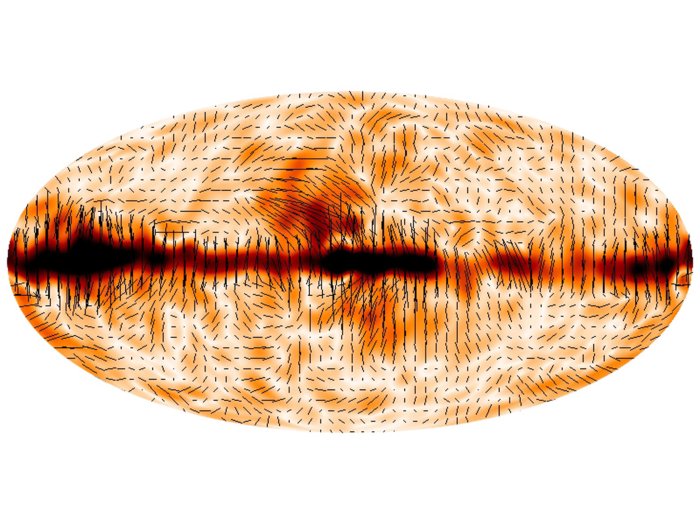
Source: science.org
Our current understanding of the cosmos, beautifully painted by the Cosmic Microwave Background (CMB), is far from a complete masterpiece. While the CMB has provided invaluable insights into the early universe, several puzzles remain, prompting exciting avenues for future research. These challenges not only refine our existing cosmological models but also push the boundaries of our understanding of fundamental physics.
The precision of CMB measurements has unveiled subtle inconsistencies with the standard cosmological model, ΛCDM (Lambda Cold Dark Matter). These discrepancies, while seemingly small, could hint at physics beyond our current comprehension. Further exploration is needed to determine if these are simply statistical fluctuations or genuine signs of new physics.
Limitations in CMB Data Interpretation
Interpreting the CMB data is not a straightforward task. Systematic errors, arising from our instruments and our understanding of foreground contamination (like dust and gas in our galaxy), can subtly affect the measurements. Accurately accounting for these systematic effects is crucial for extracting reliable cosmological information. For example, subtle variations in the polarization of the CMB can be misinterpreted if we don’t precisely model the effects of galactic dust polarization. This necessitates meticulous calibration and data analysis techniques, constantly refined through rigorous testing and validation. Moreover, the current CMB data, while incredibly detailed, still has limitations in resolution and sensitivity. Higher resolution and sensitivity will allow us to probe smaller angular scales and fainter signals, revealing finer details of the early universe.
Open Questions Regarding the Early Universe
The early universe remains shrouded in mystery. While the CMB provides a snapshot of the universe at around 380,000 years old, the period before that – the era of inflation and the very beginning – remains largely theoretical. We lack a complete understanding of the physics governing these epochs.
The nature of dark matter and dark energy, which constitute the vast majority of the universe’s energy density, remains a significant enigma. Precise measurements of CMB parameters could offer crucial clues to their properties.
Another open question is the origin of the primordial density fluctuations, the seeds of large-scale structure formation. The CMB provides a map of these fluctuations, but their exact origin and mechanism of generation are still under investigation.
Role of Future CMB Experiments
Future CMB experiments, such as the Simons Observatory and CMB-S4, are designed to address these limitations and open questions. These next-generation experiments will employ significantly improved instrumentation and observational strategies. They will achieve higher sensitivity and angular resolution, allowing for more precise measurements of CMB polarization and temperature anisotropies. This will enable us to probe smaller angular scales, revealing details of the early universe previously inaccessible.
For example, CMB-S4 aims to significantly improve the constraints on the sum of neutrino masses, a key parameter that influences the formation of large-scale structures. More precise measurements will also allow us to test the predictions of inflation with greater accuracy, potentially revealing clues about the physics at extremely high energies.
Future Research Directions, Big bang cosmic microwave background
Research into the CMB and the Big Bang theory is far from over. Future research will focus on several key areas. Improved data analysis techniques, coupled with increasingly sophisticated theoretical models, will allow us to extract maximum information from the CMB data. The development of novel techniques to reduce systematic errors and disentangle foreground contamination will be crucial. Furthermore, combining CMB data with other cosmological probes, such as large-scale structure surveys and gravitational wave observations, will provide a more comprehensive picture of the universe’s evolution. This multi-messenger approach is expected to provide powerful cross-checks and potentially uncover unexpected connections.
End of Discussion
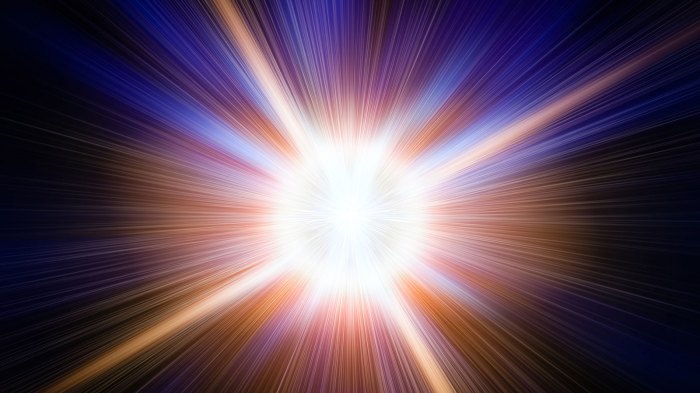
Source: hswstatic.com
The cosmic microwave background isn’t just a relic of the past; it’s a living testament to the universe’s ongoing evolution. By studying its subtle variations, we unravel the secrets of the Big Bang and gain a deeper understanding of the fundamental forces and constituents that make up our universe. Future observations promise to refine our understanding further, pushing the boundaries of our cosmological knowledge and perhaps even revealing unexpected surprises about the very fabric of reality. The journey continues, and the echoes of creation still whisper their secrets.