No fusion energy wont be limitless – No fusion energy won’t be limitless, despite the hype. While promising clean, abundant energy, the reality is far more nuanced. We’re talking massive infrastructure needs, tricky technological hurdles, and some serious environmental considerations. Think of it as a futuristic energy source with some seriously grounded problems.
This isn’t about raining on anyone’s parade; it’s about a realistic look at fusion’s potential and its limitations. From the sheer amount of energy required to build and maintain these reactors to the scarcity of crucial fuel sources, the path to limitless fusion is paved with significant challenges. Let’s dive into the nitty-gritty, shall we?
Resource Constraints in Fusion Energy Production
While fusion energy holds immense promise as a clean and virtually limitless energy source, the reality is far more nuanced. Achieving commercially viable fusion power faces significant hurdles, many stemming from resource limitations and the sheer engineering challenge involved. Let’s delve into the practical constraints that could hinder the widespread adoption of fusion energy.
Deuterium and Tritium Availability
Deuterium, a heavy isotope of hydrogen, is readily available in seawater. However, tritium, another hydrogen isotope crucial for many fusion reactions, is far rarer. While it can be extracted from lithium, the process is energy-intensive and adds to the overall energy balance. The current global supply of tritium is insufficient to fuel a large-scale fusion power plant network. Furthermore, the environmental impact of lithium mining and processing needs careful consideration, potentially offsetting some of the environmental benefits of fusion. The efficient breeding of tritium within a reactor, a process that often requires careful design and neutronics management, remains a key technological challenge.
Energy Requirements for Fusion Reactor Construction and Maintenance, No fusion energy wont be limitless
Building and maintaining a fusion reactor is an enormously energy-intensive undertaking. The construction phase alone requires vast amounts of energy for material production, manufacturing, and transportation. The reactors themselves are incredibly complex machines, demanding specialized materials that are often expensive and difficult to produce. Moreover, the operational phase necessitates substantial energy input for magnetic field generation, plasma heating, and cooling systems. The energy needed to sustain the fusion reaction itself is a significant factor, and even small inefficiencies in energy conversion can dramatically impact the net energy gain.
Energy Input versus Energy Output
Different fusion reactor designs exhibit varying energy input-to-output ratios. While theoretical models suggest high energy gains, achieving these gains in practice remains a significant challenge. For example, the International Thermonuclear Experimental Reactor (ITER) project, aiming to demonstrate the scientific and technological feasibility of fusion energy, requires a massive energy input for its operation. While ITER is not designed for net energy production, it’s a crucial step towards developing future fusion power plants with improved energy efficiency. Early fusion reactor designs may require substantially more energy to operate than they produce, leading to a negative net energy output. Future designs will need to improve this ratio considerably before fusion becomes a truly viable energy source.
Fusion Reactor Component Lifespan and Replacement Costs
The lifespan and replacement costs of fusion reactor components represent a significant economic challenge. The intense heat, neutron bombardment, and harsh operating conditions within the reactor core lead to material degradation and component failure. Replacing components, especially those within the reactor core, is an extremely complex and expensive undertaking. The table below provides estimated lifespans and replacement costs for some key components – these are estimates based on current technology and projections, and actual figures may vary considerably depending on the specific reactor design and operating conditions.
Component | Estimated Lifespan (Years) | Estimated Replacement Cost (USD Billions) | Notes |
---|---|---|---|
First Wall | 2-5 | 0.5 – 1.0 | Subject to high neutron flux and thermal stress. |
Breeding Blanket | 5-10 | 1.0 – 2.0 | Crucial for tritium breeding; complex design and material challenges. |
Magnets | 10-20 | 2.0 – 5.0 | Large, expensive components requiring specialized materials and maintenance. |
Divertor | 2-5 | 0.5 – 1.5 | Handles plasma exhaust; exposed to extreme heat and erosion. |
Technological Hurdles and Economic Viability: No Fusion Energy Wont Be Limitless
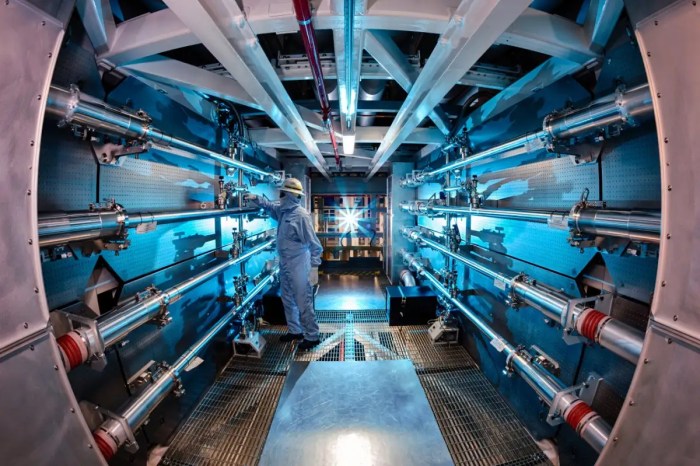
Source: newscientist.com
Let’s be real, folks: even fusion energy, that holy grail of limitless power, has its limits. The resources needed for its creation and maintenance aren’t exactly infinite, much like Link’s seemingly endless supply of courage in the upcoming Tears of the Kingdom, as discussed in this fascinating interview: tears of the kingdom link smells fujibayashi aonuma interview.
So, while it’s a massive leap forward, we shouldn’t fall into the trap of thinking any energy source is truly limitless. Sustainable practices are still key, even with fusion.
Fusion energy, while promising a clean and virtually limitless power source, faces significant technological and economic hurdles before it can become a viable alternative to existing energy sources. The path to commercial fusion power is paved with challenges that require substantial breakthroughs in materials science, plasma physics, and engineering. Overcoming these obstacles will determine whether fusion energy can truly deliver on its potential.
Plasma Confinement Challenges
Maintaining a stable plasma at the extreme temperatures and pressures required for fusion is incredibly difficult. Plasma, a superheated state of matter composed of ions and electrons, tends to expand and cool rapidly. Current approaches, such as magnetic confinement using tokamaks or stellarators, aim to contain the plasma using powerful magnetic fields. However, even with sophisticated designs, instabilities can arise, leading to energy loss and disruptions that can damage the reactor. Achieving and maintaining the necessary confinement time—the duration the plasma remains hot and dense enough for fusion reactions to occur—remains a major technological challenge. For example, ITER, the world’s largest tokamak, is designed to achieve a plasma confinement time of several hundred seconds, a significant improvement over previous experiments, but still a fraction of the time needed for sustained energy production. Further advancements in plasma control and magnetic field configurations are essential to improve confinement and enhance the efficiency of fusion reactors.
Material Limitations Under Extreme Conditions
Fusion reactors operate under incredibly harsh conditions. The intense heat and neutron bombardment generated during fusion reactions pose significant challenges to materials used in reactor construction. The reactor vessel and other components must withstand temperatures exceeding 100 million degrees Celsius and intense neutron fluxes that can cause material degradation and embrittlement over time. Developing materials that can tolerate these extreme conditions for extended periods is crucial for the long-term operation and safety of fusion reactors. Current research focuses on advanced materials such as tungsten alloys, silicon carbide composites, and liquid metals, which exhibit superior heat resistance and radiation tolerance compared to traditional materials. However, these materials are still under development, and their long-term performance under fusion reactor conditions needs further investigation.
Economic Competitiveness of Fusion Energy
The projected costs of fusion energy production are a significant concern. Building and operating fusion reactors requires substantial investments in research, development, and infrastructure. ITER, for example, is a multi-billion dollar international project, highlighting the immense capital investment needed for even experimental reactors. While the cost of fusion energy is expected to decrease as technology matures, it is still uncertain whether it can compete economically with existing energy sources like solar, wind, and natural gas, especially in the near term. Detailed cost-benefit analyses, incorporating factors like plant lifetime, fuel costs, and decommissioning expenses, are crucial to determine the long-term economic viability of fusion energy. For instance, comparing the levelized cost of electricity (LCOE) for fusion power plants with that of existing renewable energy sources is a key aspect of determining its market competitiveness. Significant technological advancements and economies of scale are needed to bring down the cost of fusion energy to a level that makes it commercially attractive.
Technological Breakthroughs Needed for Economic Viability
Achieving economically competitive fusion energy requires several key technological breakthroughs.
- Improved Plasma Confinement: Developing advanced confinement techniques to significantly increase the plasma confinement time and reduce energy losses.
- Radiation-Hard Materials: Creating materials capable of withstanding the intense heat and neutron bombardment within a fusion reactor for extended operational lifetimes.
- High-Efficiency Energy Conversion: Developing more efficient methods for converting the kinetic energy of fusion products into electricity.
- Reduced Reactor Complexity and Cost: Designing simpler, more compact, and less expensive fusion reactor designs.
- Advanced Tritium Breeding: Developing efficient tritium breeding blankets to ensure a sustainable supply of fuel for fusion reactors.
Environmental Impact and Waste Management
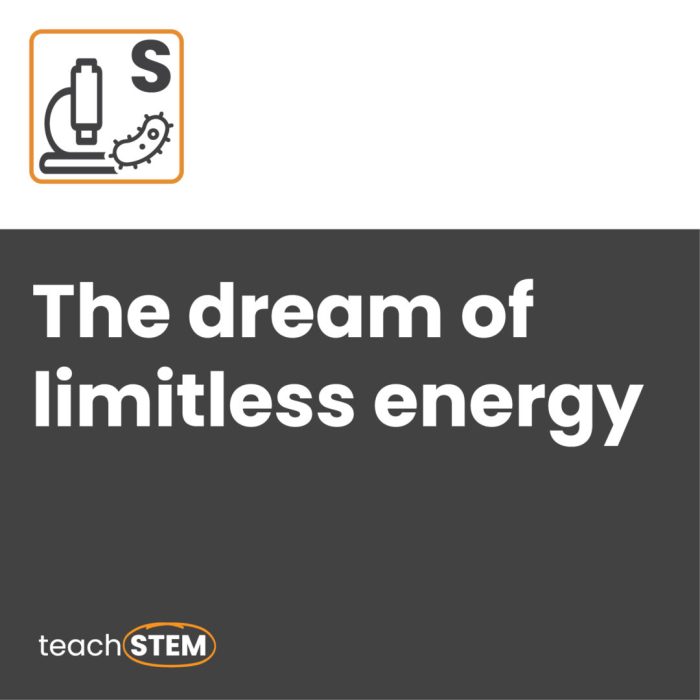
Source: com.au
Fusion energy, while promising a clean energy future, isn’t without its environmental considerations. While significantly cleaner than fossil fuels, the process isn’t entirely benign, and careful management of potential risks is crucial for its widespread adoption. We need to assess not only the operational impacts but also the long-term consequences of waste disposal and potential accidents.
Potential Environmental Risks During Reactor Operation and Decommissioning
Fusion reactors, despite not directly emitting greenhouse gases, pose some environmental challenges. Tritium, a key component of the fusion fuel, is radioactive and its accidental release could contaminate the environment. The high temperatures and pressures within the reactor necessitate robust containment structures, and the failure of these structures could lead to the release of radioactive materials. Furthermore, the decommissioning process of a fusion reactor, involving the handling and disposal of activated materials, presents significant logistical and environmental challenges. These challenges require stringent safety protocols and advanced waste management strategies throughout the reactor’s lifecycle. The scale of these challenges is comparable to those faced in the nuclear fission industry, demanding equally rigorous solutions.
Radioactive Waste Management in Fusion Energy
Fusion reactors produce radioactive waste, though the volume and type differ significantly from fission reactors. The primary radioactive waste is generated from the activation of structural materials within the reactor due to neutron bombardment. This activated material requires careful handling and long-term storage, similar to the management of high-level waste from fission reactors. However, the overall volume of radioactive waste from fusion is anticipated to be considerably less than that produced by fission, and the radioactivity is typically of shorter duration. Strategies for waste management involve various techniques including direct disposal in deep geological repositories, recycling of reusable materials, and transmutation to reduce the long-term radioactivity. The specific approach will depend on the materials used in the reactor design and the type of waste generated.
Long-Term Effects of Tritium Release
Tritium, a radioactive isotope of hydrogen, is a crucial fuel component in fusion reactions. Its release into the environment, even in small quantities, is a concern due to its potential to accumulate in biological systems. Tritium can be incorporated into water molecules, becoming part of the hydrological cycle and potentially affecting aquatic life and humans through ingestion or absorption through the skin. Long-term effects can include genetic damage and an increased risk of cancer. Strict containment measures and robust monitoring systems are crucial to mitigate the risks associated with tritium release. While the half-life of tritium is relatively short (12.3 years), its potential for bioaccumulation necessitates stringent environmental protection strategies.
Comparison of Environmental Impact of Fusion Energy with Other Energy Sources
Energy Source | Greenhouse Gas Emissions | Radioactive Waste | Other Environmental Impacts |
---|---|---|---|
Fusion | Very Low | Low to Moderate (depending on reactor design) | Tritium release, potential for accidents |
Fossil Fuels (Coal, Oil, Gas) | Very High | Negligible | Air and water pollution, habitat destruction, greenhouse gas effect |
Nuclear Fission | Low | High | Risk of accidents, long-term waste disposal challenges |
Solar and Wind | Very Low | Negligible | Land use impacts, visual impacts, intermittent energy supply |
The Role of Scientific Breakthroughs and Unexpected Challenges
Fusion energy, while promising limitless clean power, faces a complex interplay of scientific breakthroughs and unforeseen challenges. The path to a functioning fusion reactor isn’t a straight line; it’s a winding road riddled with potential obstacles that could significantly delay or even derail the entire endeavor. Unexpected scientific hurdles, coupled with the sheer complexity of the technology, mean that the timeline for widespread fusion power remains highly uncertain.
The pursuit of fusion energy hinges on our ability to control and harness the immense power of nuclear fusion. However, the plasma – the superheated state of matter at the heart of fusion reactions – is notoriously unstable. Even minor fluctuations can disrupt the fusion process, leading to reduced energy output or even catastrophic reactor failure. Similarly, the materials used to construct fusion reactors must withstand incredibly harsh conditions, including extreme temperatures and neutron bombardment. Unforeseen material degradation could compromise the reactor’s integrity and safety, necessitating costly repairs or even complete reactor redesign.
Unforeseen Scientific Obstacles and Their Impact
The delicate balance required for sustained fusion reactions means that seemingly minor scientific discoveries can have major consequences. For example, the discovery of a previously unknown plasma instability could render existing reactor designs ineffective, requiring years of research and development to find a solution. Similarly, unforeseen material limitations – such as unexpected levels of radiation damage or erosion – could necessitate the use of entirely new materials, adding significant time and cost to the project. The ITER project, for example, has encountered several unforeseen challenges related to material science and plasma control, highlighting the unpredictable nature of fusion research. These challenges demonstrate the iterative nature of the process; progress isn’t always linear.
The Impact of Material Degradation and Plasma Instabilities
Material science plays a crucial role in fusion energy. The reactor components must withstand extreme temperatures, neutron bombardment, and high magnetic fields. Unexpected material degradation, such as faster-than-predicted erosion or embrittlement, could lead to premature component failure, requiring costly replacements and potentially lengthy downtime. Similarly, plasma instabilities, which are inherently difficult to predict and control, can disrupt the fusion process, reducing energy output and potentially damaging reactor components. The unpredictable nature of these phenomena makes it difficult to accurately estimate the lifespan and efficiency of fusion reactors. Research into advanced materials and improved plasma control techniques is crucial for mitigating these risks.
Examples of Minor Technological Issues with Significant Impacts
Even seemingly minor technological hurdles can significantly impact the timeline and feasibility of fusion power. For instance, the development of high-efficiency superconducting magnets is crucial for confining the plasma. Unexpected difficulties in manufacturing or operating these magnets could lead to significant delays and increased costs. Another example could be the development of robust and reliable diagnostic tools for monitoring the plasma. Without accurate and real-time diagnostics, it is impossible to optimize the fusion process, potentially limiting the efficiency and lifespan of the reactor. These are not theoretical concerns; such issues have arisen in various fusion projects.
Hypothetical Scenario: An Unexpected Challenge
* The Scenario: Imagine a scenario where, after decades of research and billions of dollars invested, a new type of plasma instability is discovered just as a prototype fusion reactor is nearing completion. This instability, previously unknown and unforeseen, renders the reactor’s confinement system ineffective at the high energy levels required for sustained fusion.
* The Impact: This discovery would necessitate a complete redesign of the reactor’s core components, potentially adding another decade or more to the development timeline and billions of dollars to the overall cost. The viability of the project, already facing economic and political pressures, could be severely compromised. This would not only delay the commercialization of fusion power but could also significantly reduce investor confidence, potentially halting further development altogether. It underscores the inherent risks associated with large-scale scientific endeavors.
Societal and Political Factors Influencing Fusion Energy Development
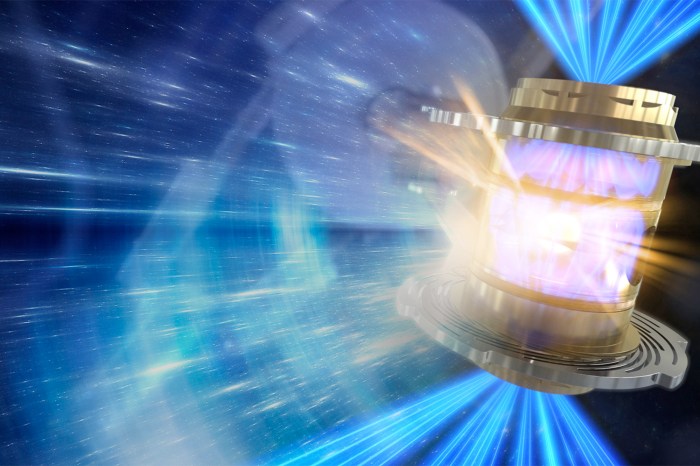
Source: nypost.com
The path to harnessing fusion energy isn’t solely a scientific endeavor; it’s deeply intertwined with societal and political landscapes. Government policies, international collaborations, and public perception all play crucial roles in determining the speed and success of fusion energy development. Understanding these factors is vital for a realistic assessment of fusion’s future.
Government funding and policy are the lifeblood of large-scale scientific projects like fusion research. The level of investment directly impacts the scale of research, the speed of technological advancements, and the overall feasibility of commercialization. Furthermore, supportive regulatory frameworks are necessary to streamline the development and deployment of fusion power plants, mitigating potential risks and ensuring public safety.
Government Funding and Policy’s Influence on Fusion Energy Research
Significant government investment is the cornerstone of fusion research. For instance, the ITER project, a multinational collaboration, relies heavily on contributions from participating nations. Changes in government priorities or budget cuts can drastically affect research timelines and outcomes. A consistent, long-term commitment from governments is crucial, as fusion research requires decades of sustained effort. Furthermore, policies that incentivize private sector involvement, such as tax breaks or grants, can accelerate innovation and commercialization. The success of other large-scale scientific projects, like the Human Genome Project, demonstrates the importance of sustained government support and clear policy frameworks.
International Collaboration in Overcoming Technological Hurdles
The complexities of fusion technology necessitate international collaboration. Sharing resources, expertise, and data across borders accelerates progress and reduces the individual financial burden on participating nations. The ITER project serves as a prime example, uniting scientists and engineers from around the globe to build the world’s largest experimental fusion reactor. This collaborative approach is essential for tackling the immense technological challenges inherent in fusion energy development, including materials science, plasma physics, and engineering. The pooling of resources and expertise through international partnerships allows for a more rapid and efficient path to a working fusion reactor.
Potential Social and Political Obstacles to Widespread Fusion Energy Adoption
Despite its potential benefits, widespread adoption of fusion energy faces significant social and political obstacles. Public perception and acceptance are crucial; concerns about safety, cost, and potential environmental impacts need to be addressed through transparent communication and robust risk assessment. Furthermore, competition for resources and potential conflicts over the distribution of fusion energy could arise. Regulatory hurdles, including licensing and safety standards, need to be carefully considered to ensure the responsible development and deployment of this powerful technology. The potential for geopolitical tensions, stemming from unequal access to fusion technology or its economic benefits, also needs to be addressed proactively.
Hypothetical Timeline of Societal Impacts of Successful Fusion Energy Development
The successful development of fusion energy would have profound and multifaceted societal impacts. A hypothetical timeline illustrates these potential consequences:
- 2040-2060: Initial demonstration of commercially viable fusion power plants. This period would see a gradual shift towards fusion energy, with initial deployments in energy-intensive industries and select regions. Increased energy security and a reduction in reliance on fossil fuels are anticipated, but widespread adoption is still limited by high initial costs.
- 2060-2080: Fusion energy becomes increasingly cost-competitive with fossil fuels and other renewable energy sources. Widespread adoption accelerates, leading to significant reductions in greenhouse gas emissions and improved air quality in urban areas. However, economic disruption in the fossil fuel industry and potential job losses in related sectors become significant concerns. International cooperation in fusion technology deployment is crucial, addressing concerns about equity and access.
- 2080-2100: Fusion energy becomes the dominant global energy source. A significant reduction in global warming is observed, with positive impacts on climate change mitigation. However, the long-term societal and economic consequences of this energy transition, including potential resource scarcity related to fusion reactor materials, need continued monitoring and management.
Visual Representation of Energy Production and Consumption
Understanding the energy flow within a fusion reactor and its overall efficiency is crucial for assessing its viability as a power source. Visual representations can help bridge the gap between complex scientific principles and public comprehension, offering a clearer picture of the energy journey from input to output. This section will explore such visualizations, focusing on energy losses and comparing fusion’s scale and land use with other energy generation methods.
Visualizing the energy flow in a fusion reactor requires a multi-stage diagram. Imagine a flowchart starting with the massive input of energy required to heat the plasma to the necessary temperature – this could be depicted as a large, brightly colored arrow representing the initial energy input, perhaps labeled “External Heating (e.g., Ohmic heating, Neutral Beam Injection)”. This initial energy input is substantial, and a significant portion is lost immediately as heat to the reactor walls. This loss could be represented by a smaller, diverging arrow labeled “Heat Loss to Reactor Walls”. Next, the fusion reactions themselves are depicted – a central, vibrant section representing the fusion process generating energy. This energy, however, isn’t directly usable. It’s primarily in the form of high-energy neutrons. A large arrow representing this neutron flux leads to a section depicting the “Blanket” – a critical component that absorbs the neutrons and converts their kinetic energy into heat. Here, another loss occurs, represented by a smaller arrow labeled “Neutron Leakage”. The heat generated in the blanket then flows to a conventional steam turbine cycle, similar to those used in fission reactors. This can be represented by arrows showing the conversion of heat energy into mechanical energy and finally into electricity, with smaller arrows branching off at each stage to depict losses due to friction, heat transfer inefficiencies, and energy conversion limitations. The final output, usable electricity, is represented by a comparatively smaller arrow, illustrating the overall efficiency of the process, highlighting the considerable energy losses throughout the entire cycle. This comprehensive visual would clearly show the energy journey, emphasizing the significant energy losses at each stage and the relatively small proportion of initial input energy that ultimately converts into usable electricity.
Scale and Size Comparison of Fusion Power Plants
A fusion power plant’s scale would be visually represented by comparing it to existing power plants. A schematic could depict a typical coal-fired power plant, a nuclear fission plant, and a hypothetical fusion plant side-by-side. The fusion plant, while potentially more compact than a coal plant in terms of land area occupied by the reactor itself, would likely still require considerable infrastructure for safety systems, cooling towers, and energy distribution, possibly occupying a similar overall footprint to a fission plant. The schematic could use scaled diagrams, highlighting the relative sizes of each plant’s core components and the surrounding infrastructure, providing a clear visual comparison of the scale of each technology. For instance, a simple comparison could show that while the fusion reactor core itself might be smaller than a fission reactor core, the necessary supporting infrastructure, such as tritium processing and plasma containment systems, could lead to a similar overall size.
Land Use Comparison for Different Energy Production Methods
A compelling illustration could be a comparative map showcasing the land use requirements of different energy generation methods. A simplified representation could use colored areas to represent the land needed per unit of energy produced. For example, a large area might be assigned to represent the land required for a coal mine and power plant, with a smaller area for a solar farm, a wind farm, and a fusion power plant. The relative sizes of these areas would visually communicate the land-use efficiency of each technology. While the fusion reactor itself may have a smaller footprint than, say, a solar farm of equivalent output, the supporting infrastructure and safety considerations could impact the overall land use. This visual would clearly show the comparative land use requirements, allowing for an immediate understanding of the spatial impact of each energy production method. The map could also incorporate labels indicating the energy output per unit area for each technology, providing a comprehensive comparison of land-use efficiency.
Epilogue
So, while the dream of limitless clean energy from fusion is incredibly appealing, the truth is a bit more complex. The path to practical, widespread fusion power is a long and winding road, fraught with technological, economic, and environmental hurdles. It’s not a matter of *if* but *when* – and whether it will ever truly be limitless. The future of energy is exciting, but let’s keep our feet firmly planted on the ground.