Quantum sound: It sounds like something straight out of a sci-fi movie, right? But the reality is even more mind-bending. Forget what you think you know about sound waves; we’re diving deep into the quantum realm, where the rules are rewritten and sound behaves in ways that defy classical physics. Prepare to have your auditory perception shattered as we explore the bizarre and beautiful world of quantum acoustics, from entanglement’s impact on sound propagation to its potential to revolutionize medical imaging and quantum computing.
This journey into the quantum world of sound will unravel the mysteries behind its unique properties. We’ll examine how quantum mechanics alters our understanding of sound waves, contrasting it with classical physics and exploring phenomena like superposition and entanglement. We’ll also delve into potential applications across various fields, from improving medical imaging techniques to advancing quantum computing. Get ready for a sonic adventure unlike any other.
Defining Quantum Sound
So, you’ve heard whispers of quantum sound, but what exactly *is* it? It’s not just your average, everyday sound amplified to eleven. Instead, it delves into the bizarre and beautiful world of quantum mechanics, where the rules of the macroscopic world we know and love go out the window. We’re talking about the quantum realm’s impact on sound waves, a realm where things get seriously weird.
Quantum mechanics fundamentally alters our understanding of energy and its interactions, and sound is fundamentally about energy transfer. Classical physics describes sound as a continuous wave, but at the quantum level, sound manifests as discrete packets of energy called phonons, analogous to photons in light. These phonons interact with matter in quantized ways, leading to effects not seen in the classical world.
Quantum Mechanics Principles Relevant to Sound
The core principles of quantum mechanics relevant to quantum sound include quantization of energy, wave-particle duality, and quantum superposition. Quantization means that energy exists in discrete packets, not as a continuous flow. Wave-particle duality suggests that phonons, while behaving like waves, also exhibit particle-like properties. Superposition allows phonons to exist in multiple states simultaneously until measured. These principles lead to unusual acoustic phenomena that defy classical explanations.
Classical vs. Quantum Descriptions of Sound Waves
The differences between classical and quantum descriptions of sound waves are significant. Classically, sound is a continuous wave described by its amplitude, frequency, and wavelength. Quantum mechanically, sound is described by the properties of phonons, including their momentum, energy, and wave function. The classical description is accurate for macroscopic sound waves, while the quantum description is necessary to understand sound at the atomic and molecular level.
Physical Systems Exhibiting Quantum Effects on Sound
Quantum effects on sound are most pronounced in systems where the energy scales involved are comparable to the energy of individual phonons. Examples include:
* Superfluids: In superfluids, the absence of viscosity allows phonons to propagate with minimal dissipation, leading to observable quantum phenomena. The lack of resistance allows sound to travel incredibly efficiently.
* Crystals at Low Temperatures: At extremely low temperatures, the thermal vibrations of atoms in crystals are suppressed, allowing quantum effects on phonons to become more apparent. The reduced thermal noise allows for clearer observation of the quantum behavior of sound.
* Quantum Materials: Certain materials exhibit unique electronic and vibrational properties due to their quantum nature, impacting how sound propagates through them. These materials can exhibit unusual acoustic properties stemming from their quantum nature.
Comparison of Classical and Quantum Sound Properties
Property | Classical Description | Quantum Description | Example |
---|---|---|---|
Nature of Sound | Continuous wave | Discrete phonons | A vibrating guitar string vs. phonon propagation in a crystal lattice |
Energy | Continuous range of values | Quantized energy levels (nhν, where n is an integer, h is Planck’s constant, and ν is the frequency) | Sound intensity in a concert hall vs. phonon energy in a superfluid |
Propagation | Described by wave equation | Governed by quantum field theory | Sound traveling through air vs. phonon scattering in a solid |
Interaction with Matter | Absorption, reflection, refraction | Quantized absorption and emission of phonons | Sound damping in a room vs. phonon-electron interaction in a metal |
Quantum Phenomena Affecting Sound Propagation
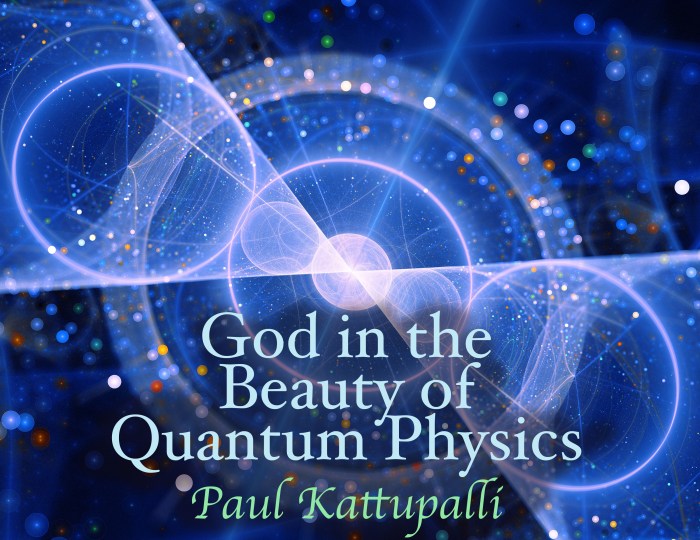
Source: doctorpaul.org
Quantum sound, a mind-bending concept, can feel like a scam itself sometimes, right? Before you invest in any “quantum sound” technology promising untold riches, remember to do your research – seriously, check out this article on how to not get scammed out of 50000 to avoid losing a hefty sum. Understanding the potential pitfalls helps you navigate the sometimes murky world of quantum sound applications and invest wisely.
The seemingly classical world of sound waves actually intersects with the bizarre realm of quantum mechanics in fascinating ways. While we don’t typically experience quantum effects in our everyday auditory experiences, at a fundamental level, quantum phenomena subtly influence how sound travels and interacts with its environment. Understanding these interactions opens up exciting possibilities for new technologies and a deeper understanding of the universe itself.
Quantum entanglement, superposition, and tunneling – these aren’t just abstract concepts confined to theoretical physics; they have the potential to significantly impact sound propagation, albeit often in subtle and challenging-to-detect ways.
Quantum Entanglement’s Influence on Sound Wave Behavior
Imagine two sound waves, perfectly correlated, entangled like two particles in a quantum experiment. A change in one wave, even at a significant distance, would instantaneously affect the other. While perfectly demonstrating entanglement with macroscopic sound waves is currently beyond our technological capabilities due to the decoherence challenges, the theoretical possibility suggests that if we could create and maintain such entangled sound states, we could potentially transmit information faster than the speed of sound, or achieve unprecedented levels of precision in acoustic sensing. The challenge lies in maintaining the quantum coherence of the sound waves over long distances and preventing environmental noise from destroying the entanglement. This area requires further exploration into the creation of robust entangled acoustic systems.
Quantum Superposition’s Effect on Sound Transmission
Quantum superposition, where a system exists in multiple states simultaneously, could theoretically impact sound transmission. A sound wave, in principle, could exist in a superposition of different frequencies or amplitudes at once. This would lead to highly complex interference patterns and potentially allow for novel ways to manipulate and control sound waves. For instance, a sound wave in superposition could simultaneously travel through multiple paths, potentially leading to unexpected interference effects and influencing the overall sound profile. However, observing this phenomenon with macroscopic sound waves faces significant experimental hurdles due to the rapid decoherence of such superimposed states.
Quantum Tunneling and Sound Propagation
Quantum tunneling, where a particle passes through a potential barrier even if it doesn’t have enough energy to overcome it classically, could affect sound propagation through certain materials. Imagine a sound wave encountering a barrier, such as a dense material. While classically the sound wave might be largely reflected or absorbed, quantum tunneling suggests a small probability of the wave “tunneling” through the barrier. This effect is likely to be extremely small for macroscopic sound waves and typical materials, but it becomes more relevant at smaller scales or with specific material properties that facilitate tunneling. This phenomenon could be investigated by analyzing sound transmission through nanoscale structures or metamaterials designed to enhance quantum tunneling effects.
A Hypothetical Experiment Demonstrating Quantum Effects on Sound Interference
A hypothetical experiment could involve creating two entangled phonon sources (phonons are the quanta of sound). These sources would emit sound waves in a controlled environment, minimizing external noise and decoherence. The waves would be directed towards a detector where their interference pattern is measured. By manipulating the entanglement between the sources, we could observe changes in the interference pattern, demonstrating the impact of entanglement on the sound wave behavior. The experiment would require sophisticated equipment for generating and controlling entangled phonons, maintaining a low-noise environment, and precisely measuring the interference patterns. The success of such an experiment would hinge upon overcoming significant technological challenges related to creating and maintaining entangled phonon states and isolating them from environmental noise.
Technological Applications of Quantum Sound
The exploration of quantum sound, while still in its nascent stages, holds immense promise for revolutionizing various technological fields. Its unique properties, stemming from the quantum nature of sound waves, offer the potential to surpass the limitations of classical acoustics and unlock unprecedented capabilities in areas such as medical imaging, materials science, and quantum computing. The following sections delve into specific applications, highlighting the transformative power of this emerging field.
Quantum Sound in Medical Imaging
Quantum sound offers the potential to significantly enhance medical imaging techniques. Imagine a world where non-invasive medical imaging could provide incredibly detailed, high-resolution images of the human body with unprecedented sensitivity. By leveraging the principles of quantum mechanics, specifically the wave-particle duality of phonons (sound quanta), researchers envision developing new imaging modalities that can detect subtle changes in tissue density and composition. This could lead to earlier and more accurate diagnoses of diseases, paving the way for more effective treatments. For example, quantum acoustic microscopy could achieve resolutions far beyond current capabilities, allowing for the detection of microscopic tumors or cellular abnormalities. The use of entangled phonons could further enhance image quality and reduce noise, providing even clearer and more precise diagnostic information.
Quantum Sound in Materials Science
The application of quantum sound in materials science promises breakthroughs in understanding and manipulating material properties at the atomic level. By precisely controlling and manipulating phonons, scientists could design materials with tailored acoustic properties. This includes creating materials with enhanced sound absorption, transmission, or even manipulation of sound at specific frequencies. For example, the ability to precisely control phonon interactions could lead to the development of new metamaterials with unprecedented acoustic properties, such as perfect sound absorbers or highly efficient acoustic waveguides. This could have profound implications for noise reduction technologies, improved acoustic sensors, and the development of novel acoustic devices. Furthermore, understanding phonon dynamics at the quantum level can help in designing stronger, lighter, and more durable materials for various applications.
Quantum Sound in Quantum Computing
Quantum sound offers a unique pathway for developing novel quantum computing architectures. Phonons, being quasiparticles, can exhibit quantum behavior, such as superposition and entanglement, making them potential candidates for carrying quantum information. Researchers are exploring the possibility of using phonons to create quantum bits (qubits) and build quantum circuits. The advantage of using phonons lies in their potential for long-range coherence and relatively low decoherence rates compared to other qubit platforms. This could lead to the development of more stable and scalable quantum computers. Furthermore, quantum acoustic networks, based on the manipulation of entangled phonons, could provide new avenues for quantum communication and computation. For instance, researchers could potentially utilize phonons to create quantum links between different parts of a quantum computer, facilitating efficient information transfer between qubits.
Future Applications of Quantum Sound
The potential applications of quantum sound extend far beyond the currently explored areas. The following list showcases some exciting future possibilities:
- Development of highly sensitive quantum acoustic sensors for environmental monitoring and security applications.
- Creation of quantum acoustic devices for advanced communication technologies.
- Design of novel quantum acoustic resonators for precision measurements and timekeeping.
- Application in quantum metrology for enhanced precision measurements of physical quantities.
- Exploration of quantum acoustic effects in condensed matter physics for fundamental research.
Challenges and Limitations: Quantum Sound
Harnessing the power of quantum sound is a monumental task, fraught with significant technological hurdles. The delicate nature of quantum phenomena, coupled with the inherent difficulties in manipulating and measuring them, presents a formidable challenge to researchers striving to unlock the potential of this nascent field. This section delves into the key limitations hindering the progress of quantum sound research and development.
The creation and manipulation of quantum sound require incredibly precise control over the environment. Maintaining the coherence of quantum states, crucial for observing quantum effects, is incredibly difficult due to interactions with the surrounding environment, a process known as decoherence. This is further exacerbated by the inherent difficulty in generating and detecting phonons—the quanta of sound—with sufficient precision and control. Current experimental techniques are often limited by noise, temperature fluctuations, and material imperfections, all of which can significantly impact the observation and manipulation of quantum sound.
Technological Hurdles in Creating and Manipulating Quantum Sound
Generating and controlling quantum sound requires extremely low temperatures and high levels of isolation from external disturbances. Sophisticated cryogenic systems and ultra-high vacuum chambers are necessary to minimize thermal noise and environmental interactions. Furthermore, the fabrication of high-quality materials with precisely defined properties is essential for generating and manipulating phonons with the desired characteristics. For example, creating high-quality superconducting circuits, which are often employed in quantum sound experiments, requires advanced nanofabrication techniques and meticulous quality control. The slightest imperfection in the material can drastically affect the quantum coherence of the system, hindering the observation of quantum phenomena.
Limitations of Current Experimental Techniques
Current experimental techniques for studying quantum sound are often limited by their sensitivity and resolution. Detecting the subtle quantum effects associated with phonons requires extremely sensitive measurement apparatuses, capable of distinguishing minute changes in the vibrational properties of materials. Furthermore, the spatial and temporal resolution of these techniques is often insufficient to fully characterize the complex dynamics of quantum sound propagation. For instance, measuring the precise position and momentum of a phonon is extremely challenging due to the Heisenberg uncertainty principle. This principle dictates that there’s a fundamental limit to the precision with which both the position and momentum of a particle can be known simultaneously. As a result, researchers often rely on indirect measurements and sophisticated theoretical models to infer the properties of quantum sound.
Feasibility of Different Approaches to Generating Quantum Sound
Several approaches are being explored to generate quantum sound, each with its own set of advantages and disadvantages. One promising approach involves using superconducting circuits to create and manipulate artificial phonons. This method offers a high degree of control over the properties of the phonons, but it is technologically demanding and requires specialized fabrication techniques. Another approach involves using ultracold atomic gases to generate and study quantum sound. This method allows for the creation of highly controlled environments, but it is experimentally challenging and requires sophisticated laser cooling techniques. The feasibility of each approach depends on factors such as the desired level of control over the quantum system, the available experimental resources, and the specific scientific question being addressed. For example, the superconducting circuit approach might be more suitable for studying specific quantum phenomena, while the ultracold atomic gas approach might be better suited for investigating fundamental aspects of quantum mechanics.
Difficulties in Measuring and Interpreting Quantum Sound Phenomena
Measuring and interpreting quantum sound phenomena present significant challenges due to the inherently weak nature of the signals and the complex interactions between phonons and other degrees of freedom in the system. The weak signal-to-noise ratio often makes it difficult to distinguish genuine quantum effects from background noise. Furthermore, the interpretation of the experimental data requires sophisticated theoretical models and advanced data analysis techniques. Ambiguities in the interpretation of experimental results can arise from the complexity of the system under study, making it difficult to isolate and characterize specific quantum effects. This necessitates the development of new theoretical frameworks and advanced experimental techniques to overcome these limitations and provide a more comprehensive understanding of quantum sound phenomena.
Quantum Sound and Information Processing
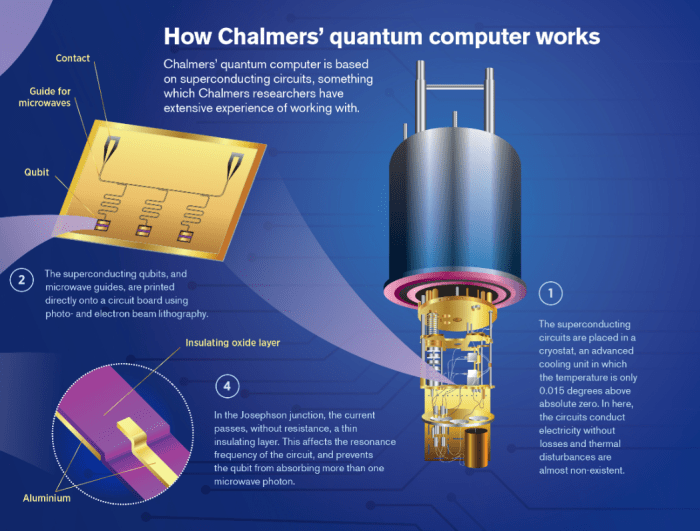
Source: 202q-lab.se
Harnessing the bizarre properties of quantum mechanics, the field of quantum sound opens up exciting possibilities for revolutionizing information processing. By manipulating sound waves at the quantum level, we can potentially encode and transfer information with unprecedented security and efficiency, surpassing the limitations of classical approaches. This exploration delves into the potential of quantum sound for creating faster, more secure, and more powerful computing and communication systems.
Quantum sound, with its inherent quantum properties like superposition and entanglement, offers a unique avenue for encoding information. Instead of using classical bits representing 0 or 1, quantum sound allows for the encoding of qubits, which can represent 0, 1, or a superposition of both simultaneously. This dramatically increases the information carrying capacity of a single sound wave. Furthermore, the entanglement of multiple sound waves can create highly correlated quantum states, providing a robust foundation for secure quantum communication protocols.
Quantum Sound Encoding and Transfer
The fundamental principle lies in manipulating the quantized vibrational modes of a medium, such as phonons in a crystal lattice, to represent qubits. These phonons, the quanta of sound, can be manipulated using techniques like quantum control of acoustic resonators or superconducting circuits. Information is encoded in the state of these phonons, and the transfer occurs through the propagation of the sound wave itself. For example, a specific phonon configuration could represent a particular qubit state, and the propagation of this configuration through the medium would constitute the transfer of information. This differs significantly from classical sound-based communication, which relies solely on the amplitude and frequency of sound waves.
Improved Signal Processing with Quantum Sound
Quantum sound offers the potential for significant advancements in signal processing. The ability to create and manipulate entangled phonon states allows for the development of highly sensitive sensors and detectors. For instance, a quantum sensor based on entangled phonons could detect incredibly subtle changes in the environment, far exceeding the capabilities of classical sensors. Moreover, quantum algorithms could be implemented using quantum sound, leading to exponential speedups in certain computational tasks compared to classical signal processing methods. This could revolutionize fields such as medical imaging, materials science, and geophysical exploration.
Conceptual Model of Quantum Sound in Quantum Communication
Imagine a system where two distant parties, Alice and Bob, each possess a quantum acoustic resonator. These resonators are carefully prepared in an entangled state, meaning their phonons are correlated in a way that defies classical explanation. Alice encodes information onto her resonator by manipulating the state of her phonons. Due to entanglement, this manipulation instantly affects the state of Bob’s resonator, allowing for the instantaneous transfer of information, even across vast distances. This represents a secure quantum communication channel, as any attempt to intercept the information would inevitably disrupt the entangled state, alerting Alice and Bob to the intrusion. While instantaneous communication is a simplification for illustrative purposes, the inherent speed advantages over classical communication are noteworthy.
Theoretical Limitations of Quantum Sound for Quantum Information Processing
While the potential is immense, challenges remain. Maintaining the coherence of quantum states, crucial for preserving quantum information, is a significant hurdle. Environmental noise and interactions with the medium can lead to decoherence, causing the loss of quantum information. The distance over which quantum sound can propagate while maintaining coherence is currently limited. Furthermore, the development of efficient and scalable quantum acoustic devices for generating, manipulating, and detecting quantum sound is a technological challenge requiring significant advancements in materials science and nanotechnology. Finally, the development of robust error correction codes tailored to the specific noise characteristics of quantum sound systems is essential for achieving reliable quantum information processing.
Illustrative Examples
Quantum sound, while still largely theoretical, offers tantalizing possibilities across various scientific and technological domains. Let’s explore a couple of hypothetical scenarios to better grasp its potential impact. These examples, while fictional, are grounded in established principles of quantum mechanics and materials science.
Quantum Sound in a Solid-State System
Imagine a meticulously crafted silicon crystal, doped with precisely controlled amounts of phosphorus. This material, chosen for its well-understood phonon properties and ability to support coherent phonon propagation, is cooled to near absolute zero. At these cryogenic temperatures, the thermal noise is significantly reduced, allowing for the observation of quantum phenomena. We introduce a focused laser pulse, precisely tuned to excite a specific vibrational mode within the crystal lattice. This excitation generates a coherent phonon wave packet – a quantum sound wave – that propagates through the material. The key quantum effect observed is the wave-particle duality of the phonons. By carefully measuring the arrival times and intensities of the phonon wave packet at various points within the crystal, we can observe interference patterns, a hallmark of wave-like behavior. Simultaneously, the detection of individual phonon excitations demonstrates the particle nature of the quantum sound. The precise control over the phonon wave packet, enabled by the quantum nature of the system, allows for the investigation of fundamental aspects of phonon-phonon interactions and energy transport at the quantum level. This experiment provides direct evidence of quantum sound and opens the door for the development of quantum devices based on phonon manipulation.
Quantum Sound for Advanced Medical Imaging
Consider a novel medical imaging technique leveraging the properties of quantum sound. Instead of using electromagnetic radiation like X-rays or ultrasound, this method employs carefully engineered pulses of coherent phonons. These phonons, generated using a specialized device, are focused on the target tissue. The phonons interact differently with various tissue types based on their density and elastic properties. The backscattered phonons, carrying information about the internal structure of the tissue, are then detected with extreme precision. This approach offers several advantages over traditional methods. First, the high frequency of the phonons allows for significantly improved spatial resolution, enabling the visualization of much smaller structures. Second, the coherent nature of the quantum sound allows for better signal-to-noise ratio, leading to clearer and more detailed images. Third, because phonons interact weakly with biological tissues compared to X-rays, this technique promises reduced radiation exposure to the patient. For example, this technology could be revolutionary in early cancer detection, providing highly sensitive images of subtle changes in tissue structure that might otherwise be missed by conventional methods. The improved resolution could also be crucial in neuroimaging, allowing for the visualization of fine neural structures and processes.
Conclusive Thoughts
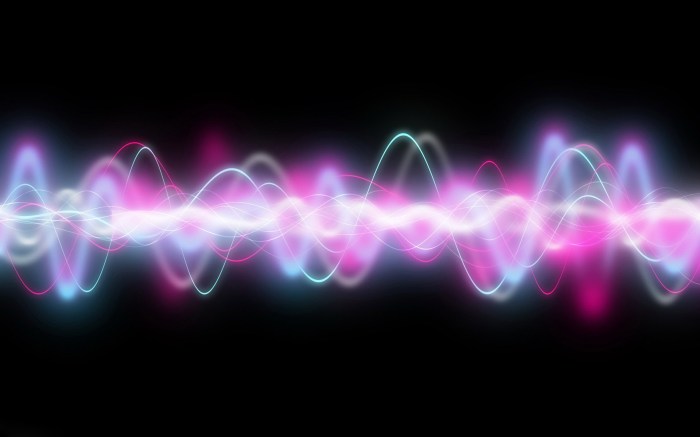
Source: clipart-library.com
So, there you have it: a glimpse into the fascinating and still largely unexplored world of quantum sound. While significant technological hurdles remain, the potential applications are breathtaking. From revolutionizing medical diagnostics to unlocking the secrets of quantum computing, quantum sound promises a future filled with sonic innovation. The journey has just begun, and the echoes of this quantum revolution are only just starting to be heard.